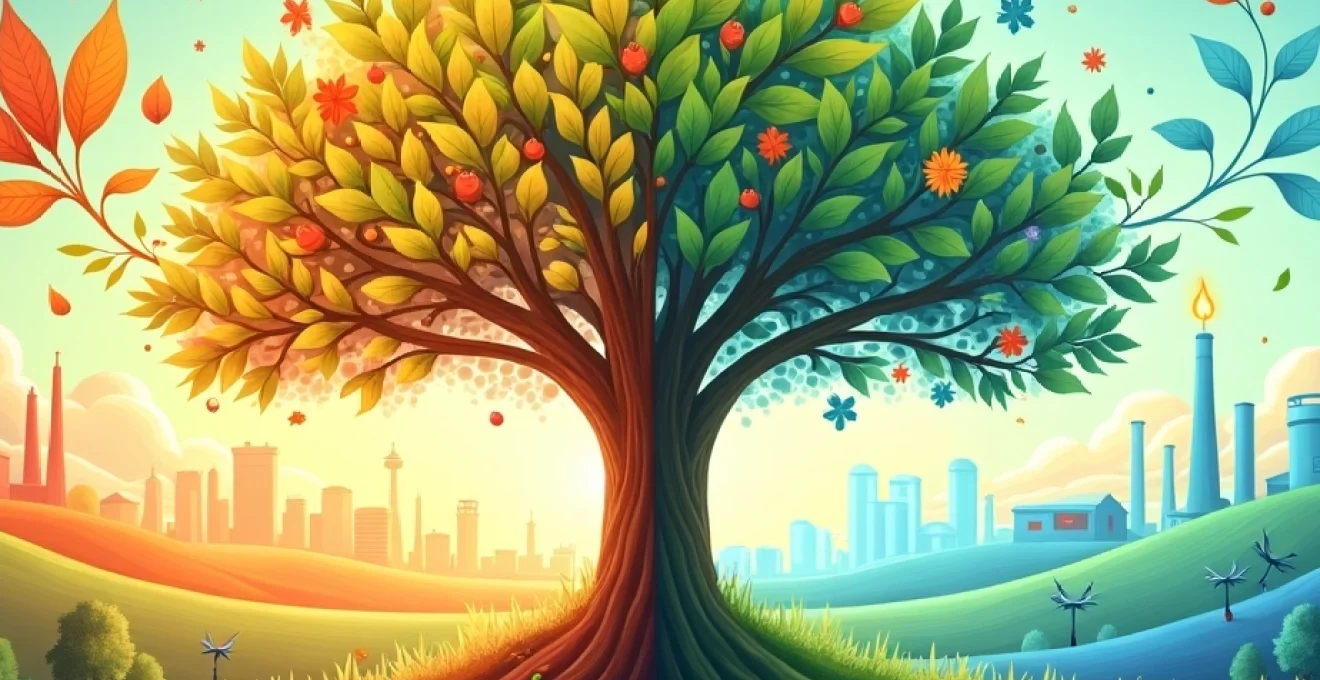
As the world grapples with climate change and seeks sustainable alternatives to fossil fuels, biomass energy has emerged as a promising solution in the renewable energy sector. This versatile energy source, derived from organic materials, is reshaping our approach to power generation and waste management.
Fundamentals of biomass energy conversion technologies
Biomass energy conversion technologies encompass a wide array of processes that transform organic matter into usable forms of energy. These technologies can be broadly categorized into thermochemical and biochemical conversion methods. Each approach offers unique advantages and is suited to different types of biomass feedstocks.
Thermochemical conversion processes include combustion, gasification, and pyrolysis. These methods use heat and controlled chemical reactions to break down biomass into energy-rich products. On the other hand, biochemical conversion processes like anaerobic digestion and fermentation rely on microorganisms to break down organic matter and produce biofuels.
The choice of conversion technology depends on factors such as the type of biomass available, the desired end product, and the scale of operation. For instance, direct combustion is often used for woody biomass in large-scale power plants, while anaerobic digestion is more suitable for wet organic waste in smaller, decentralized facilities.
Feedstock diversity and preprocessing methods for biomass
One of the key strengths of biomass energy is its feedstock diversity. From agricultural waste to dedicated energy crops, the range of materials that can be used for biomass energy production is vast. This diversity not only enhances the resilience of biomass energy systems but also allows for regional customization based on locally available resources.
Agricultural residues: corn stover and rice husk utilization
Agricultural residues like corn stover and rice husks represent a significant untapped resource for biomass energy. These materials, often left in fields after harvest, can be collected and processed to produce energy. Corn stover, consisting of stalks, leaves, and cobs, is particularly abundant in regions with large-scale corn production. Rice husks, a byproduct of rice milling, are widely available in rice-producing countries.
Preprocessing of these residues typically involves collection, size reduction, and drying. Advanced techniques like pelletization can improve the handling and combustion properties of these materials. By utilizing agricultural residues, biomass energy production can provide additional income for farmers while reducing waste and environmental impact.
Forestry byproducts: sawdust and bark processing techniques
The forestry industry generates significant amounts of byproducts that can be used for biomass energy. Sawdust and bark, often considered waste in traditional lumber production, are valuable feedstocks for bioenergy. These materials are typically processed through techniques like screening, drying, and densification to improve their energy content and handling characteristics.
Torrefaction, a mild form of pyrolysis, is an emerging technique for preprocessing woody biomass. This process improves the energy density and hydrophobic properties of the biomass, making it more suitable for long-term storage and transportation. The resulting torrefied biomass has properties similar to coal, allowing for easier integration into existing power generation infrastructure.
Energy crops: miscanthus and switchgrass cultivation strategies
Dedicated energy crops like Miscanthus and switchgrass are increasingly being cultivated for biomass energy production. These perennial grasses offer high yields and can be grown on marginal lands, reducing competition with food crops. Cultivation strategies for these crops focus on maximizing biomass production while minimizing inputs.
Miscanthus, known for its rapid growth and high biomass yield, requires careful establishment but can provide harvests for up to 20 years. Switchgrass, native to North America, is drought-tolerant and well-adapted to a range of soil conditions. Both crops benefit from precision agriculture techniques, including optimized planting densities and nutrient management.
Municipal solid waste: sorting and preparation for energy recovery
Municipal solid waste (MSW) represents a significant opportunity for biomass energy production, particularly in urban areas. However, effective sorting and preparation are crucial to ensure the quality and safety of the feedstock. Advanced sorting technologies, including optical sorters and robotic systems, are increasingly being employed to separate organic waste from other materials.
Once sorted, the organic fraction of MSW can be preprocessed through methods like shredding and moisture control. These steps prepare the waste for energy recovery processes such as anaerobic digestion or incineration. By diverting organic waste from landfills to energy production, biomass energy systems can significantly reduce greenhouse gas emissions while generating valuable power and heat.
Thermochemical conversion processes in biomass energy
Thermochemical conversion processes form the backbone of many large-scale biomass energy systems. These methods leverage high temperatures and controlled chemical reactions to transform biomass into energy-rich products. The choice of thermochemical process depends on factors such as the desired end product, feedstock characteristics, and scale of operation.
Pyrolysis: fast and slow techniques for bio-oil production
Pyrolysis is a versatile thermochemical process that decomposes biomass in the absence of oxygen. This process can be tailored to produce different proportions of solid, liquid, and gaseous products. Fast pyrolysis, characterized by rapid heating and short residence times, maximizes the production of bio-oil. This liquid product can be used directly as a fuel or upgraded to produce transportation fuels.
Slow pyrolysis, on the other hand, involves lower temperatures and longer residence times. This approach favors the production of biochar, a solid carbon-rich material with applications in soil amendment and carbon sequestration. The choice between fast and slow pyrolysis depends on the desired product mix and the specific characteristics of the biomass feedstock.
Gasification: syngas generation and cleaning methods
Gasification converts biomass into a combustible gas mixture known as syngas. This process involves partial oxidation of the biomass at high temperatures, typically above 700°C. The resulting syngas, primarily composed of hydrogen, carbon monoxide, and methane, can be used for power generation, heat production, or as a feedstock for chemical synthesis.
Syngas cleaning is a critical step in the gasification process, as the raw gas contains impurities that can damage downstream equipment or reduce efficiency. Cleaning methods include particulate removal through cyclones and filters, tar reduction through thermal or catalytic cracking, and removal of sulfur and nitrogen compounds. Advanced cleaning technologies, such as hot gas filtration, are being developed to improve the overall efficiency of biomass gasification systems.
Torrefaction: enhancing biomass energy density
Torrefaction is a mild form of pyrolysis that improves the properties of biomass for energy applications. This process, typically carried out at temperatures between 200-300°C in an inert atmosphere, removes moisture and low-energy volatile compounds from the biomass. The result is a hydrophobic, energy-dense material with improved grindability and combustion characteristics.
The benefits of torrefaction include reduced transportation costs due to increased energy density, improved storage stability, and enhanced co-firing potential with coal. However, the technology is still in the early stages of commercial deployment, with ongoing research focused on optimizing process parameters and scaling up production.
Combustion: direct firing and co-firing with coal
Direct combustion remains the most widely used method for converting biomass to energy, particularly for heat and power generation. Modern biomass combustion systems employ advanced technologies to improve efficiency and reduce emissions. These include fluidized bed combustors, which allow for better mixing and heat transfer, and advanced air staging techniques to minimize NOx formation.
Co-firing biomass with coal in existing power plants has gained traction as a strategy to reduce carbon emissions without significant infrastructure changes. This approach typically involves blending biomass with coal at ratios of up to 20%. Co-firing can be implemented through direct co-firing, where biomass and coal are combusted together, or indirect co-firing, where biomass is gasified separately and the resulting syngas is combusted with coal.
Biochemical conversion routes for biomass utilization
Biochemical conversion processes leverage the power of microorganisms to break down biomass into valuable energy products. These methods are particularly well-suited for wet biomass feedstocks and can produce a range of biofuels and biochemicals. The key biochemical conversion routes include anaerobic digestion, fermentation, and enzymatic hydrolysis.
Anaerobic digestion: biogas production from organic waste
Anaerobic digestion is a biological process that breaks down organic matter in the absence of oxygen, producing biogas and digestate. This technology is particularly effective for treating wet organic waste streams, including food waste, agricultural residues, and sewage sludge. The resulting biogas, primarily composed of methane and carbon dioxide, can be used for heat and power generation or upgraded to biomethane for injection into natural gas networks.
Recent advancements in anaerobic digestion technology have focused on improving process stability and methane yields. These include the development of two-stage digestion systems, which separate the hydrolysis and methanogenesis stages, and the use of co-digestion strategies to optimize feedstock composition. Additionally, innovative pretreatment methods, such as ultrasonic and thermal hydrolysis, are being employed to enhance the biodegradability of recalcitrant feedstocks.
Fermentation: ethanol and butanol synthesis from cellulosic materials
Fermentation is a key process in the production of liquid biofuels from biomass. While first-generation bioethanol production from sugar and starch crops is well-established, there is growing interest in cellulosic ethanol production from lignocellulosic biomass. This approach utilizes agricultural residues, forestry waste, and dedicated energy crops, avoiding competition with food production.
The production of cellulosic ethanol involves several steps, including pretreatment to break down the complex structure of lignocellulose, enzymatic hydrolysis to convert cellulose to sugars, and fermentation of these sugars to ethanol. Recent research has focused on developing consolidated bioprocessing (CBP) approaches, where cellulase production, cellulose hydrolysis, and fermentation are combined in a single step, potentially reducing costs and improving efficiency.
Enzymatic hydrolysis: breaking down complex carbohydrates
Enzymatic hydrolysis is a critical step in the conversion of lignocellulosic biomass to fermentable sugars. This process uses specialized enzymes, primarily cellulases and hemicellulases, to break down the complex carbohydrates in biomass into simple sugars. The efficiency of enzymatic hydrolysis significantly impacts the overall economics of cellulosic biofuel production.
Advances in enzyme engineering and production have led to significant reductions in enzyme costs and improvements in hydrolysis efficiency. Novel approaches, such as the use of designer cellulosomes – engineered enzyme complexes that mimic natural cellulose-degrading systems – show promise for further enhancing the effectiveness of enzymatic hydrolysis. Additionally, the integration of enzymatic hydrolysis with other conversion processes, such as simultaneous saccharification and fermentation (SSF), can improve overall process efficiency.
Integration of biomass in power generation systems
The integration of biomass into power generation systems represents a significant opportunity to reduce carbon emissions and enhance energy security. This integration can take various forms, from dedicated biomass power plants to co-firing in existing coal-fired facilities. The choice of integration strategy depends on factors such as biomass availability, existing infrastructure, and policy incentives.
Dedicated biomass power plants, typically ranging from 1 to 50 MW in capacity, offer the advantage of optimized design for biomass feedstocks. These plants often employ advanced combustion technologies like fluidized bed boilers, which can handle a wide range of biomass types and qualities. Combined heat and power (CHP) configurations are particularly attractive for biomass plants, as they can achieve overall efficiencies of up to 80% by utilizing waste heat for industrial processes or district heating.
Co-firing biomass with coal in existing power plants has gained traction as a cost-effective approach to reducing carbon emissions. This strategy can be implemented through direct co-firing, where biomass is mixed with coal and combusted in the same boiler, or indirect co-firing, where biomass is gasified separately and the resulting syngas is combusted with coal. Co-firing ratios typically range from 5% to 20% on an energy basis, with some advanced systems achieving higher percentages.
The integration of biomass gasification with combined cycle gas turbines (BIOMASS-IGCC) represents an advanced power generation concept. This approach offers high electrical efficiencies, comparable to those of natural gas combined cycle plants, while utilizing renewable biomass feedstocks. However, the technology is still in the early stages of commercialization, with ongoing efforts to improve gas cleaning and system reliability.
Environmental impact and sustainability metrics of biomass energy
As biomass energy continues to expand, it is crucial to assess its environmental impacts and sustainability. While biomass offers potential benefits in terms of carbon emissions reduction and waste management, concerns have been raised about land use changes, water consumption, and biodiversity impacts. Comprehensive life cycle assessments (LCAs) are essential to understand the true environmental footprint of biomass energy systems.
Carbon neutrality debate: life cycle assessment approaches
The carbon neutrality of biomass energy has been a subject of intense debate. While biomass is often considered carbon-neutral due to the carbon absorption during plant growth, this simplification can overlook important factors such as land use changes, fertilizer use, and processing emissions. Accurate assessment of biomass carbon impacts requires comprehensive LCAs that consider the entire supply chain, from feedstock production to end-use.
Recent LCA studies have highlighted the importance of considering temporal dynamics in carbon accounting for biomass systems. The concept of carbon debt and payback time is particularly relevant for forestry-derived biomass, where the time required for carbon resequestration can span decades. Advanced LCA methodologies, such as dynamic LCA and consequential LCA, are being developed to better capture these temporal and market-mediated effects.
Land use changes: balancing food, fuel and ecosystem services
The expansion of biomass energy production raises concerns about potential competition with food production and impacts on ecosystem services. Direct land use change, such as converting food cropland to energy crops, can have immediate impacts on food security and biodiversity. Indirect land use change, where biomass production displaces other activities to new areas, is more challenging to quantify but potentially significant.
Strategies to mitigate land use impacts include focusing on marginal or degraded lands for energy crop cultivation, utilizing agricultural and forestry residues, and promoting agroforestry systems that integrate food and fuel production. Advanced land use modeling techniques, such as spatially explicit life cycle assessment, are being developed to better understand and manage these complex land use dynamics.
Water footprint: consumption and treatment in biomass energy systems
Water consumption is a critical consideration in biomass energy systems, particularly in regions facing water scarcity. The water footprint of biomass energy varies significantly depending on the feedstock and conversion technology. Irrigated energy crops can have high water requirements, while the use of agricultural residues or rainfed crops may have minimal additional water impacts.
In biomass conversion processes, water is consumed for feedstock preparation, process cooling, and flue gas treatment. Advanced water management strategies, such as closed-loop cooling systems and wastewater recycling, can significantly reduce the water footprint of biomass energy plants. Additionally, the integration of biomass energy systems with wastewater treatment facilities offers opportunities for synergistic water and energy management.
Biodiversity impacts: monoculture risks and mitigation strategies
The expansion of energy crop cultivation raises concerns about potential negative impacts on biodiversity, particularly if large-scale monocultures replace diverse natural habitats. However, when properly managed, biomass production can also offer opportunities for habitat creation and biodiversity enhancement.
Mitigation strategies to promote biodiversity in biomass systems include using diverse crop mixes, implementing wildlife-friendly harvesting practices, and maintaining habitat corridors. The concept of multifunctional landscapes, where biomass production is integrated with other ecosystem services, offers a promising approach to balancing energy production with biodiversity conservation.
Research into the ecological impacts of different biomass feedstocks and production systems is ongoing, with a focus on developing sustainability indicators and certification schemes. These efforts aim to provide a framework for assessing and promoting biodiversity-friendly biomass production practices.
Integration of biomass in power generation systems
The integration of biomass into existing power generation systems presents both opportunities and challenges. As the energy landscape evolves, biomass is increasingly being viewed as a complementary fuel source that can enhance the flexibility and sustainability of power grids.
One of the most promising approaches is the development of hybrid power plants that combine biomass with other renewable sources. For instance, biomass-solar hybrid systems can leverage the dispatchability of biomass to complement the intermittency of solar power. These integrated systems can provide more reliable and consistent power output, addressing one of the key challenges of renewable energy integration.
Another area of focus is the optimization of biomass co-firing in coal power plants. Advanced co-firing technologies, such as separate injection systems and dedicated biomass gasifiers, are being developed to increase the biomass-to-coal ratio beyond traditional limits. These innovations not only reduce carbon emissions but also extend the operational life of existing coal infrastructure during the transition to cleaner energy sources.
The concept of biomass-to-power microgrids is gaining traction, particularly in rural and off-grid areas. These systems combine local biomass resources with smart grid technologies to provide reliable and sustainable power to communities. By utilizing locally available feedstocks and incorporating energy storage solutions, biomass microgrids can enhance energy security and promote rural development.
As we look to the future, the integration of biomass in power generation systems will likely be characterized by increased digitalization and smart control strategies. Advanced monitoring and predictive maintenance systems will optimize biomass fuel handling and combustion processes, improving efficiency and reducing downtime. Furthermore, the use of artificial intelligence and machine learning algorithms will enable better forecasting of biomass availability and demand, facilitating smoother integration with other renewable energy sources.
Environmental impact and sustainability metrics of biomass energy
The environmental impact and sustainability of biomass energy systems are complex and multifaceted issues that require careful consideration. As the biomass industry continues to grow, it is crucial to develop comprehensive metrics and assessment tools to ensure that biomass energy contributes positively to our sustainability goals.
Carbon neutrality debate: life cycle assessment approaches
The carbon neutrality of biomass energy remains a contentious issue in scientific and policy circles. While the basic premise of carbon neutrality is based on the carbon cycle of plant growth and combustion, the reality is far more complex. Life Cycle Assessment (LCA) approaches have become increasingly sophisticated in capturing the full range of emissions associated with biomass energy systems.
Recent advancements in LCA methodologies include the incorporation of time-dependent carbon accounting. This approach recognizes that the carbon debt incurred when biomass is harvested and burned is only repaid over time as new plants grow and sequester carbon. Depending on the type of biomass and management practices, this carbon payback period can range from a few years for fast-growing crops to several decades for forest biomass.
Another important development in LCA approaches is the consideration of indirect land-use change (ILUC) effects. ILUC occurs when biomass production displaces other land uses, potentially leading to deforestation or conversion of grasslands elsewhere. While challenging to quantify, ILUC can significantly impact the overall carbon balance of biomass energy systems.
Land use changes: balancing food, fuel and ecosystem services
The expansion of biomass energy production has raised concerns about competition with food production and impacts on ecosystem services. Striking the right balance between these competing land uses is crucial for the sustainable development of the biomass sector.
One promising approach is the concept of multifunctional landscapes, where biomass production is integrated with food production and ecosystem conservation. Agroforestry systems, for example, can combine woody biomass production with food crops or livestock grazing, maximizing land use efficiency while providing multiple ecosystem services.
Advanced land-use modeling techniques are being developed to optimize biomass production while minimizing negative impacts. These models consider factors such as soil quality, water availability, biodiversity hotspots, and existing land use patterns to identify the most suitable areas for sustainable biomass cultivation.
Water footprint: consumption and treatment in biomass energy systems
The water footprint of biomass energy systems varies widely depending on feedstock type, cultivation practices, and conversion technologies. As water scarcity becomes an increasingly pressing issue in many regions, understanding and managing the water impacts of biomass energy is crucial.
Innovative water management strategies are being implemented across the biomass supply chain. In feedstock production, precision irrigation techniques and drought-resistant crop varieties can significantly reduce water consumption. At the conversion stage, closed-loop water recycling systems and advanced wastewater treatment technologies are being employed to minimize freshwater withdrawal and environmental impacts.
The water-energy nexus presents opportunities for synergistic solutions. For instance, the integration of biomass energy systems with wastewater treatment plants can provide mutual benefits. Biomass can be used to power the treatment process, while treated wastewater can be used for biomass cultivation or process cooling, creating a circular water-energy system.
Biodiversity impacts: monoculture risks and mitigation strategies
The potential impact of large-scale biomass production on biodiversity is a significant concern, particularly when it involves monoculture plantations. However, with proper planning and management, biomass cultivation can be designed to support and even enhance biodiversity.
Diversification strategies are key to mitigating biodiversity risks. Mixed plantations that combine multiple species can provide a range of habitats and support greater biodiversity than monocultures. Additionally, the strategic placement of wildlife corridors and habitat patches within biomass plantations can maintain connectivity for wildlife movement.
Adaptive management approaches are being developed to monitor and respond to biodiversity impacts over time. These involve regular biodiversity assessments, flexible management practices, and stakeholder engagement to ensure that biomass production evolves in harmony with local ecosystems.
The development of sustainability certification schemes specifically addressing biodiversity conservation in biomass production is gaining momentum. These schemes provide a framework for assessing and promoting biodiversity-friendly practices, helping to ensure that the growth of the biomass sector contributes positively to global conservation efforts.